ATOMIC BACKGROUND
AND EXAFS OF GASEOUS HYDRIDES OF Ge, As, Se, AND Br
Related
publications: R.
Prešeren, A.
Kodre, I. Arčon, M. Borowski,
J. Synchrotron Rad., 8, (2001), p. 279-281 (reprint)
Introduction
Detailed analysis
of absorption spectra reveals fingerprints of collective excitations
of the atom, mostly as tiny resonances
and jumps above
K and L absorption edges. Although most of the experimental evidence
has been accumulated from experiments on noble gases (Schaphorst
et al., 1993), the results are relevant also for the EXAFS analysis.
The collective excitations occupy the same spectral region as the
EXAFS signal and thus comprise its non-structural part, the atomic
absorption background (AAB). In routine EXAFS work, the background
is conveniently separated from the structural signal in the transform
space: it is reconstructed by a spline from the low wavenumber
components. However, the sharp features of the collective excitations
occupy
a broad wavenumber interval, so that some leakage into the structural
signal is inevitable (Frahm et al., 1984;
Kochur et al., 1986; Kodre et al., 1994; Chaboy et al., 1994; Kodre
et al., 1995;
Filipponi,
1995; Filipponi & Di Cicco, 1995; D'Angelo et al., 1996; Kodre
et al., 1997; Kodre et al., 1999; Padežnik Gomilšek et al., 1999).
For precision EXAFS analysis, an independently determined AAB is
thus required. The basic assumption, however, is that of transferability:
the atomic background depends mainly on intra-atomic dynamics and
not on the environment of the atom (Kodre
et al., 2000).
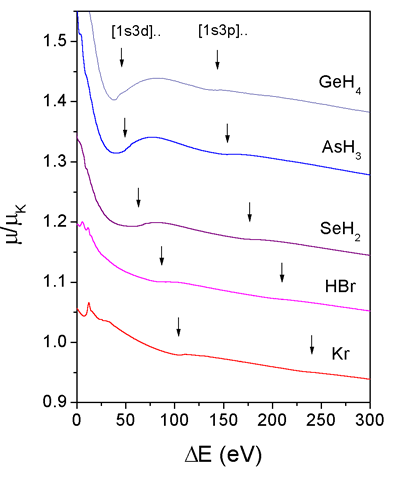 |
Fig.
1. Normalised K edge absorption spectra of GeH4,
AsH3, SeH2, and HBr. The spectrum of Kr is added for comparison.
A relative energy scale with origin at the K edge is used.
Thresholds for [1s3d] and [1s3p] multielectron excitations
are indicated by arrows. The spectra are displaced vertically
for clarity. |
The scarce data on the independent AAB have been collected in several
ways. A direct measurement is only possible on monatomic gases.
Beside noble gases, hardly interesting for EXAFS, some metal
vapors have
been studied (Filipponi et al., 1993;
Prešeren et al., 1996; Kodre et al.,
1997; Arčon et al., 1997; Prešeren et al., 1999; Prešeren & Kodre,
1999-a). By a reverse analysis, AAB can be obtained as a remainder
of an EXAFS signal after the structural signal of sample with a well-known
(or a very simple) structure has been removed. This technique has
been exploited in AXAFS (atomic EXAFS) investigations (Holland
et al., 1978; Rehr et al., 1994). By combining two or more
samples, the need for a well-known structure is dispensed - in
this way, AAB
of the series
of 4p elements have been determined (Padežnik
Gomilšek et al., 1999-a).
In some cases, an iterative procedure on a single sample succeeded
without additional information (Li et al.,
1992; D'Angelo et al., 1993; D'Angelo et al., 1995; Bridges
at al., 1995; D'Angelo et al., 1996).
There is also a semiempirical approach, where AAB is constructed
from atomic binding energies and cross sections (Di
Cicco, 1995; Di Cicco et al., 1996; Arčon et al., 1997). It
is simplified by the fact that only multielectron excitations of
core
+ first subvalence electrons
contribute to the EXAFS AAB. The excitations involving valence
electrons are limited to within 30 eV of the edge in the XANES
region, while
those involving deeper shells appear more than 1000 eV above the edge.
In the present
study, we demonstrate that gaseous hydrides of 4p elements Ge, As,
Se, Br can be used in determining the AAB
with the same precision
as that of elemental monatomic samples, exploiting the low-noise
gas absorption spectroscopy and the fact that the small structural
signal
of scattering on hydrogen can be determined ab initio. Bromine hydride
has been studied in this way before (D'Angelo
et al., 1993). The
non-negligible scattering contribution of hydrogen neighbors
has already been demonstrated
in an experiment on germane (Bouldin et al.,
1981).
Experiment
Germane (GeH4),
arsine (AsH3), hydrogen selenide (SeH2), and hydrogen bromide (HBr)
have been synthesised for the purpose and sealed
in 12 cm long glass cells with kapton windows. For the aggressive
HBr,
a
cell with thin (0.3 mm) glass windows had to be used, with subsequent
tenfold increase in the noise level. The gas pressure in the
cells was chosen to ensure absorption length md ~ 2 at the
K edge.
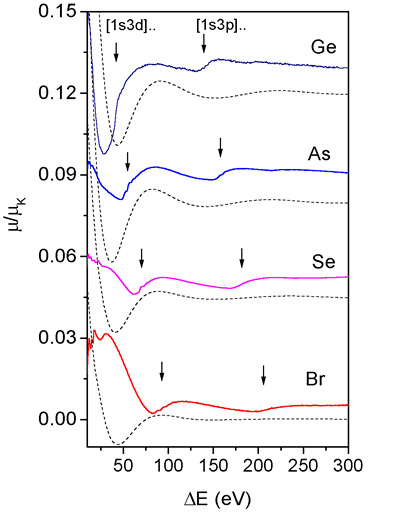 |
Fig.
2. The decomposition of the hydride absorption
spectra of Fig. 1 into the ab initio calculated EXAFS signal
(dashed line) and AAB (solid line). Average linear
trend is subtracted from the AAB spectra for better comparison with the EXAFS
spectra. A relative energy scale with origin at the K edge is used. Multielectron
excitation [1s3d] and [1s3p] shake-up edges in the AAB spectra are indicated
by arrows. The spectra are displaced vertically for clarity. |
The experiments were performed at the beamline BM 29 of the European
Synchrotron Radiation Facility ESRF in Grenoble, France and at the
beamline ROEMO2 (X1.1) in Hamburger Synchrotronstrahlungslabor HASYLAB
at Deutschen Elektronen -Synchrotron DESY (Hamburg, Germany). At both
beamlines a Si(311) fixed-exit double-crystal monochromator was used
with 0.8 eV and 1.5 eV resolution at 12 keV, respectively. Harmonics
were effectively eliminated by detuning the monochromator crystal using
a stabilization feedback control. Ionization cells filled with argon
were used to detect incident and transmitted flux of the monochromatic
X-ray beam through the sample.
The absorption spectra were recorded in 0.5 eV energy steps with an
integration time of 2 s/step. Ten experimental runs were superimposed
to improve the signal-to-noise ratio. Exact energy calibration was
established with the simultaneous absorption measurements on the Pt
metal foil and from a measurement on Kr with a well defined K edge.
The absorption cells were equipped with a side chamber into which the
gas could be frozen in situ and thus removed from the beam, to obtain
a precision reference measurement of the window transmission and energy
dependence of detector efficiency.
Results and discussion
The above-edge
region of the absorption spectra is shown in Fig. 1. Notably, there
is hardly any recognizable structural signal,
the spectra
are all similar to the absorption spectrum of Kr, added below for
comparison. They reveal two distinct absorption edges which can,
in analogy with
Kr, be attributed to multielectron excitations involving 3d and
3p electrons. Another edge, involving 3s excitation, can be
discerned
at higher energies. The identification of the edges is confirmed
by Dirac-Fock estimates of the excitation energies.
The structural signal of the hydrides can be constructed from
known scattering amplitudes and phases (Rehr
et al., 1992; Stern et al.,
1995). The width of the hydrogen neighbor shell can be calculated
from the spectrum of molecular vibrations, which, due to the simple
geometry
of the molecules, allows exact treatment (Cyvin,
1968; Greenwood & Earnshaw,
1984). In this way, the structural signal is constructed entirely ab
initio: the best-fit determination of the EXAFS parameters which would
be unreliable in view of the prevalence of the AAB in the experimental
spectrum, is completely avoided. We can see (Fig. 2) that the structural
signal is not really negligible: in a curious coincidence, however,
the waves of the EXAFS signal follow the rise of the two absorption
edges of the AAB so that the oscillatory component of the spectrum
remains inconspicuous.
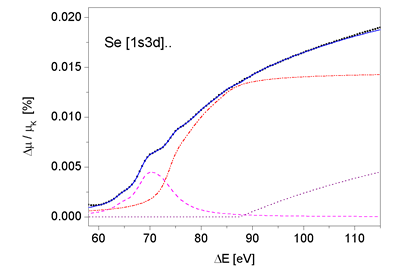 |
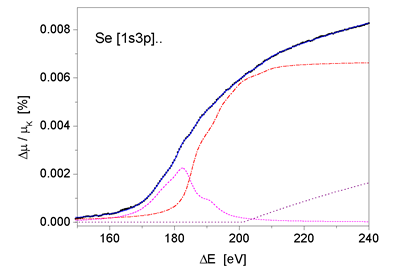 |
Fig.
3. Multiplet structure of 1s3d and 1s3p MPE features
in SeH2 AAB. Dots - experiment; contributions of atomic double
excitation
channels: resonances - (dashed line), shake-up (dash-dot line)
and shake-off (dotted line) channels. Sum of individual MPE channels
- (solid line). |
The comparison of the AAB in Fig. 2 with the earlier data from
solid samples of the same set of elements (Padežnik
Gomilšek et al., 1999-a) shows more than a tenfold
improvement in accuracy. In the earlier set, the noise of the
results comprises mostly the unresolved
high-wavenumber structural components while in the present
case we believe the
noise at the level of 2×10^(-5) in As and Se data is limited
to the detector
statistics. The high quality of the experimental data shows,
for the
first time, fine structure of the AAB features (Fig. 3). In a
detailed analysis, the features are completely resolved into
contributions
of atomic double excitation resonant, shake-up and shake-off
channels (Prešeren, 2000), with
an essential modification due to the molecular
coupling of final states. The important point, however, is that
the AAB can be described by the dynamics of the atom, and that
apart
from
details of multiplet structure which show fingerprints of the
molecule, a transferable AAB is defined with sufficient precision
even for
most sensitive EXAFS analysis (see also Kodre et al., 2000).
The fine structure of the AAB features can also be used to estimate
the contribution of AXAFS, a long-wave interference pattern arising
from the scattering at the atom boundary (Rehr
et al., 1994).
It has been proposed as an alternative, or at least complementary,
interpretation of AAB. Since sharp features and multiplet structures
by which the
major part of the extracted AAB signal is explained, are not
predicted
in AXAFS, its contribution in the AAB spectra of the investigated
hydrides
seems to be of minor importance.
Acknowledgment:
The study is supported by Internationales Büro des BMBF, Germany
and Ministry of Sciences and Technology, Slovenia. L. Troeger from
HASYLAB provided expert advice on X1 beamline operation. The experiment
at the BM29 beamline of ESRF was performed under proposal No. HE-375.